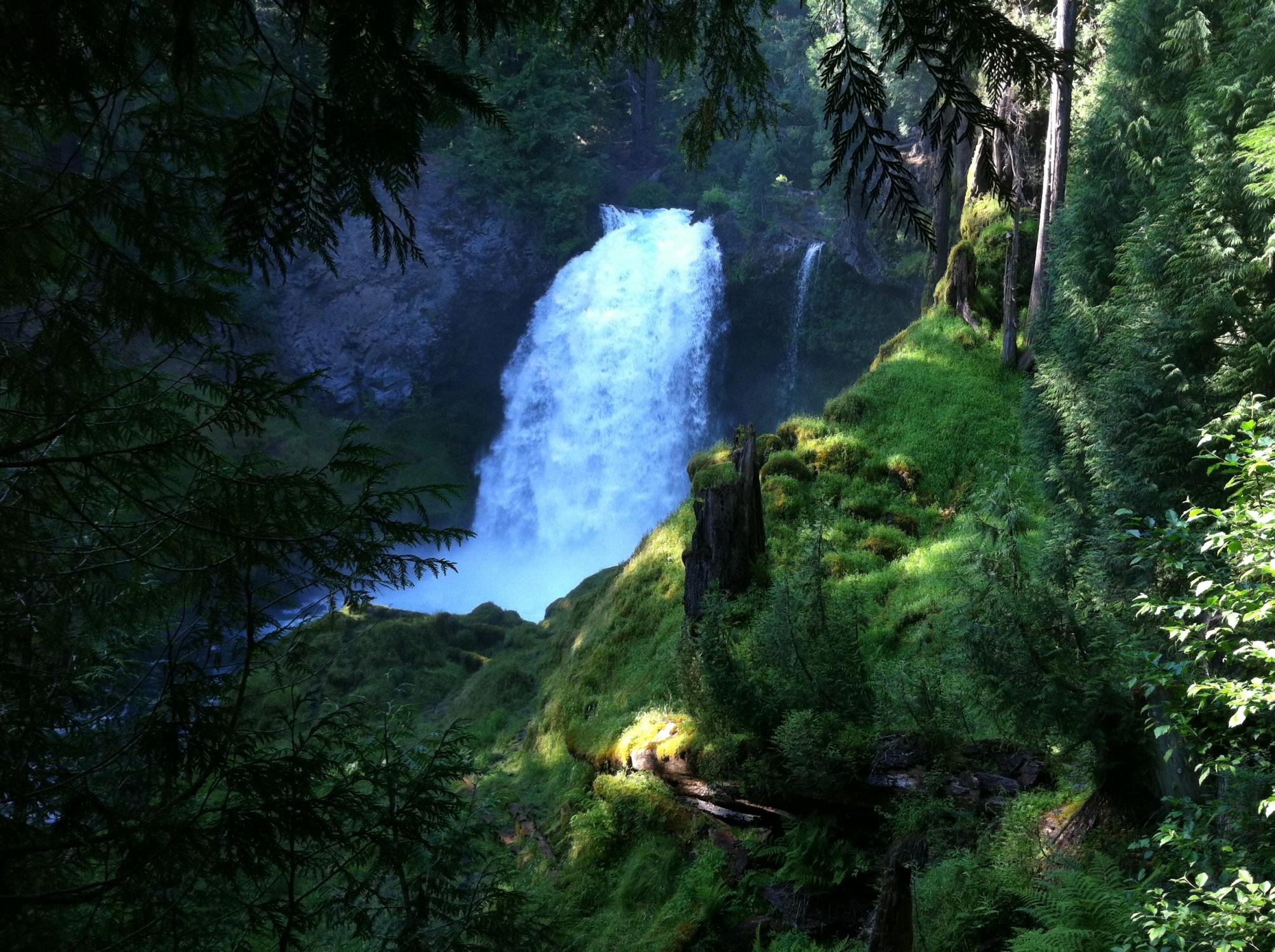
FILE- Sahalie Falls, pictured here in an undated photo, is one of several waterfalls on the 26-mile McKenzie River Trail. Researchers recently learned that the aquifer in the Cascade Mountains that feeds rivers like the McKenzie is far larger than they expected.
Jule Gilfillan / OPB
Researchers have long known there was some kind of aquifer in the Cascade Mountains that feeds rivers like the McKenzie and the Deschutes. But they never knew just how big it might be — until now, that is. A new study found that the Central Oregon aquifer holds 80 cubic kilometers of water, roughly three times the size of Nevada’s Lake Mead.
Leif Karlstrom is an associate professor of earth sciences at the University of Oregon. He led the study and joins us with more details on what the discovery could mean for the region — and why we shouldn’t view it as a water windfall.
Note: The following transcript was transcribed digitally and validated for accuracy, readability and formatting by an OPB volunteer.
Dave Miller: From the Gert Boyle Studio at OPB, this is Think Out Loud. I’m Dave Miller. Researchers have long known that there was an aquifer in the Cascade Mountains that feeds the McKenzie and Deschutes Rivers. But until recently, they didn’t know just how big it might be. A new study found that this Central Oregon aquifer holds around 80 cubic kilometers of water, meaning it’s roughly three times the size of Nevada’s Lake Mead.
Leif Karlstrom is an associate professor of earth sciences at the University of Oregon. He led this study and he joins us to talk about its implications. It’s great to have you on Think Out Loud.
Leif Karlstrom: Thanks, Dave. Thanks for having me.
Miller: What exactly did researchers already know about this aquifer?
Karlstrom: I guess we knew that it was large. We use it and the people that have been living in Central Oregon have been using it for thousands of years. We know there’s a lot of water here, over 2.5 meters of precipitation in the Western Cascades per year. And previous researchers have tried to estimate the size of the aquifer by measuring what’s coming out of the ground. So there’re these amazing spring systems, mostly on the western side, but also on the eastern side of the Cascades topographic crest. There’s a tremendous amount of water coming out of some of these spring systems. And previous researchers have used those to estimate the volume that might be stored underneath. It’s about a third of what we found.
Miller: How do you explain that? We’ll get to your methods. But it seemed like a pretty good idea – figure out how much is coming out and use that as a basis for what is in it. What do you think was wrong with that method?
Karlstrom: Certainly, nothing wrong. I think it points to one of the unique things about the Central Oregon Cascades, which is its geologic origin. I’m a volcanologist actually by training, not a hydrologist. And I came to this study from the standpoint of trying to understand the origins of this landscape. It was built by volcanoes. The Cascade mountain range was uplifted by magma, ultimately, over millions of years. And as it turns out, volcanic rocks have some rather unusual hydrogeologic properties especially when they’re young. We can talk about what that means. It means something different to be geologically young than young from a human standpoint. These young volcanic rocks tend to be highly fractured, tend to permit a lot of water seepage and flow through them. As a result, we get some fairly unusual hydrology in this region.
So it’s not a bad idea to look at the springs at all. It’s a tried-and-true method. But it doesn’t tell the whole story. And I think that’s where we saw an opening and we really tried to use a whole variety of different types of data, much of which already existed, by the way, to make a better estimate.
Miller: What kind of data did you use?
Karlstrom: This region, and the study in general, covers what we in our science call the critical zone. It’s this near-surface layer where atmospheric processes interact with the solid earth. It’s where all life exists. It’s very important. And the types of data that one uses to learn about the critical zone includes topographic data, erosion rates, weathering rates, as well as water flow through rivers, as well as groundwater.
We also used old exploration geothermal wells that were drilled in mostly the ‘70s and ‘80s, when there was some interest in potential geothermal resources in the Cascades. That ended up being a crucial data set for us because while the Cascades ended up not being very great for geothermal potential, what those wells found was a fingerprint of tremendous amounts of water seeping down through the rocks into the subsurface to great depths, over a kilometer. So we use those geothermal drill hole temperature depth profiles essentially to map out where the water is.
Miller: How is it that this data that came from the drilling, showing the relative temperatures in different areas, give you a better understanding of water?
Karlstrom: I guess the first thing to understand is what happens, in most places, when you dig a hole in the ground – and we’re talking about a deep hole here – temperatures typically go up. It’s what we call the geothermal gradient. So when you’re looking for abnormally hot regions, which this by all measures should be … There’s active volcanism at the Cascades Crest. One might think that there would be hotter than average temperatures here, and that would then imply perhaps a good place to look for geothermal resources.
That geothermal gradient was actually much, much less than expected when these holes were drilled. And there’s a wonderful term that was coined in the late ‘80s about this. They called it the rain curtain. Essentially, precipitation – largely snow but also rain – advecting or moving the heat, that would otherwise cause an increase in temperature with depth, along with it. So in other words, without the induction or the rainwater, you would normally see temperatures go up with that. But in this case, as you drill down, the temperatures remain the same as they are at the surface, or in some cases even get cooler. And that’s directly fingerprinting the downward movement of water.
Miller: Downward movement, but then it’s collecting somewhere, right? I mean, am I wrong in imagining just an enormous, giant, contiguous or continuous lake embedded in these mountains? Is that what you mean by an aquifer?
Karlstrom: No, that’s a good point. This is not a big lake that you can paddle your canoe around in. There’s a notion of active groundwater. So these are small fractures really, in which water is seeping, continuously moving over time. You can imagine a little particle of water, perhaps melting in the spring as the snowpack in the Cascades melts. It seeps into the ground and it travels dominantly vertically in the high Cascades, in the young volcanic rocks, and it seeps down and down and down. But eventually, its path turns and it will make its way out towards spring systems, largely driven by topographic gradients.
The things that we want to think about in terms of groundwater flow are both the slopes – the things that drive the groundwater flow – and then the volumes of water coming in. We know, by actually measuring isotopes in the water, roughly how long it takes for a water particle to go from the Cascade Crest to one of these springs. And it’s about seven to 10 years on average. So that’s the number to have in your head. Water’s continuously moving through the system. It’s not a big static lake. But nonetheless, it is a reservoir in the sense that at any given time, there’s quite a bit of water stored there.
Miller: That time scale is nothing geologically. But I have to say it’s really surprising to me, just in terms of what I would have guessed the adventure of a raindrop would be or a snowflake falling near the top of the mountain. Seven to 10 years before it eventually trickles down through these fissures, comes out of a spring and then eventually joins a river, joins the outside air again. It’s, to me, a very long time.
Karlstrom: Yeah, that’s right. It’s not unusual in other settings for these transit times to be much, much longer – thousands of years. And it is worth pointing out that when you see water coming out of a spring, that water is reflecting a distribution of transit times. Not all the little particles of water took seven to 10 years. Some of them actually might have taken quite a bit longer – thousands of years. So it’s actually quite a tricky problem that occupies a lot of hydrologists' time, to be honest, it is estimating these transit times.
But seven to 10 years is a very small time scale. And it’s reflective of the highly fractured nature of these rocks. It’s one of the things that makes them unusual.
Miller: So that’s one of the things. What about just the scale of this? Perhaps three times as much volume as Lake Mead. Are mountain range aquifers of this scale common?
Karlstrom: No, in some sense, this is not a new finding from the hydrogeologic perspective. We know that volcanic terrains globally, especially if they’re in wet climates, tend to host large volumes of water. Think about ocean islands. When I say that, for example, Hawaii [has] a lot of the water resources that we see in these volcanic landscapes. They are essentially similar to what we’re seeing in the Cascades. But what we have in the Cascades here is just a much larger scale. Both the particular type of post rocks, these we call mafic. It’s a particular type of volcanic rock. Not all volcanic rocks behave this way, it’s a particular kind. But we have a large volume of these, larger than average. Then we just have a tremendous amount of rainfall and precipitation, dominantly snowpack actually that’s feeding this aquifer.
The combination of those two things gives rise to a rather unusual setting, and it might be unique, in some sense, in the world. Although I will also say that people haven’t really looked. So that’s a clear opportunity for future work.
Miller: With our more chaotic climate, with the possibility of severe drought, with projections for lower snowpack – based on maybe, overall, more water falling but warmer temperatures, so less of it being stored as snowpack and maybe seeping into these fissures – what are projections for the future of this aquifer?
Karlstrom: Yeah, it’s a great question. And I don’t have the answer to that. But it’s certainly one of the things that we want to know. If we think about water resources, for example, in the West, we may first think about reservoirs, the surface water. Reservoirs that we built to manage water flow. You really need to know the whole budget, right? What’s coming in, what’s going out, all the different processes that are at play here. You certainly need to know how big it is. And we didn’t know that for the High Cascades aquifer as well as we should, and I would argue that we still don’t. Our estimates, I think, are a first step. But there’s quite a bit more work that needs to be done.
In terms of what’s likely to be seen in the future, I think you’re absolutely right, the current climate projections are that we will reduce snowpack in the Central Oregon Cascades by 60% to 90% or more by 2100. And that’s an example of what we call a climate tipping point. The mountains are only so high and if we warm the climate, there will be a point where the snow is no longer the favored mode of precipitation. So that is likely to have a pretty big impact on the rates of recharge of this aquifer. So we’d like to understand what that’s going to do.
Miller: Just briefly, we have about 45 seconds left – you mentioned you’re a volcanologist. What are the potential implications of magma, or serious seismic activity, and this much water?
Karlstrom: One of the things that we noticed when we started looking at this is that some of the youngest eruptions – and there are some eruptions in the Central Oregon Cascades that happened roughly 1,000 years ago, and certainly will happen in the future – these have a character that reflects interaction with groundwater. We call them phreatomagmatic eruptions. They tend to be more explosive than they otherwise would be. An interesting side note to this study is that by mapping out the groundwater, we’re also actually mapping out volcanic hazards. And I think that’s really important because we’d like to understand the potential for these more explosive eruptions going forward.
Miller: Leif Karlstrom, thanks so much.
Karlstrom: Thank you.
Miller: Leif Karlstrom is an associate professor of earth sciences at the University of Oregon.
Contact “Think Out Loud®”
If you’d like to comment on any of the topics in this show or suggest a topic of your own, please get in touch with us on Facebook, send an email to thinkoutloud@opb.org, or you can leave a voicemail for us at 503-293-1983. The call-in phone number during the noon hour is 888-665-5865.